-
These 'deep dives' were delivered to premium users:
-
'Interfaces: Si-based Electrodes – Sulfide Electrolytes' (10 pages)
-
'Recent Patents & Innovations in Ni-based Cathode Materials' (18 pages)
Get a quote.
-
Lithium-ion batteries – electrolytes – solid & semi-solid
-
A silicon oxide interfacial layer was deposited on a lithium metal layer (thickness: 20 μm)
via plasma-enhanced chemical vapor deposition
(PECVD). The optimal
process involves initial deposition of amorphous Si using SiH4 and Ar (1 min,
10 nm), followed by a gradual increase of the N2O : SiH4 ratio from 1 : 2 to
20 : 1 over multiple steps to form a silicon oxide layer (SiOX, X ≈ 1,
thickness: ≈25 nm).
On the lithium metal layer, an oxygen concentration gradient was
established in the silicon interfacial layer, with lower oxygen content near the metal layer
interface and higher content further away.
The electrolyte contains LiPF6 (1 mol/L) in a mixture of ethylene carbonate
(EC), propylene carbonate (PC), and dimethyl carbonate (DMC) in a 3 : 3 : 3 mass ratio. A
polyethylene (PE) separator was used.
Full cells (NMC-based positive electrodes) with silicon-based interfacial layer exhibit a capacity
retention of 98% after 500 cycles (1 C charge / discharge), while uncoated reference cells exhibit 70%
capacity retention.
This work illustrates a potentially powerful approach to achieve very promising cycling stability with lithium metal negative electrodes
by interfacing the lithium metal layer with an SiOX (X ≈ 1) interface layer with an oxygen gradient, which in turn
interfaces with the liquid electrolyte.
This patent is included in this category because it describes a concept that could be highly relevant in semi-solid or all-solid-state cells.
-
The premium version includes another two patent discussions, plus an Excel list with 50-100 commercially relevant recent patent families.
-
Lithium-ion batteries – negative electrode (excluding Li metal electrodes)
-
A porous carbon substrate (D50: 3-20 μm, pore diameter: 1-4 nm, micropore ratio:
10-100%) was placed in a chemical vapor deposition (CVD) reactor under inert
atmosphere.
Monosilane gas, NH3, and
nitrogen were introduced (flow ratio 33 : 2 : 35). The deposition was carried out
(500°C, 20 min) to form a first nitrogen-doped Si-carbon composite.
A monosilane / nitrogen mixture was introduced (flow ratio 33 : 35) for additional
silicon deposition (500°C, 20 min). This two-step process was repeated twice.
The material underwent passivation using a nitrogen and acetylene gas mixture
(flow ratio 35 : 4, 100 min). The composite was then mixed with phenolic resin (mass ratio 10 : 1)
in anhydrous ethanol and stirred (4 h).
The mixture was spray-dried (150°C) and carbonized under a NH3 atmosphere
(550°C, 4 h).
In half-cells, the material exhibits a capacity retention of 93.2% after 200 cycles
(0.1 C charge / discharge), a first cycle expansion of 20.3%, and an electronic conductivity
of 30.9 S/cm, as compared to 86.4%, 36.2%, and 19.6 S/cm respectively for a comparative
material prepared without nitrogen doping. The material exhibits a nitrogen content of 2.33 mass%, compared
to 0.83 mass% for the comparative material.
This work illustrates a multi-step process that appears to have been systematically optimized to optimize the nitrogen
content distribution in Si-carbon composite materials across Si and carbon domains.
A correlation chain was established between material and electrochemical parameters that might be highly
helpful during process up-scaling (electronic conductivity, first cycle expansion, capacity retention).
-
The premium version includes another two patent discussions, plus an Excel list with 50-100 commercially relevant recent patent families.
-
Lithium-ion batteries – positive electrode
-
MnSO4 · 5 H2O, NiSO4 ·
6 H2O, and CoSO4 · 7 H2O were mixed in a 0.4 : 0.35 : 0.25 molar ratio
to form solution 1. Na2CO3 was dissolved in water to form solution 2.
The solutions were added dropwise to a reactor under mechanical stirring (25°C, see variation of stirring rates below).
The precursor surface was coated with Na2CO3 via spray-drying (200°C, 0.3 MPa)
to achieve >75% surface coverage, followed by a heat treatment (600°C for
6 h, then 900°C for 1 h, air).
The P2-type Na-containing oxide was then ion-exchanged with a LiNO3 / LiCl molten salt
mixture (50 : 50 molar ratio, 280°C, 1 h) to obtain the O2-type Li-containing oxide.
Materials obtained with varying precursor solution mechanical stirring rates were characterized by SEM (see Figure).
With optimized stirring power (0.086 W for Example
1 [実施例1], 0.054 W for Example 2 [実施例2]), spherical particles with uniform size and
interconnected surface crystallites (<1 μm) were obtained. The moderate stirring power enables
controlled particle growth while maintaining spherical morphology.
In half-cell tests, the optimized material exhibits a 0.1 C discharge capacity of 242.0 mAh/g
and excellent rate capability with 87% capacity retention at 3 C compared to the 0.1 C rate.
At 0.5 C and 1 C rates, the capacity retentions are 96% and 94%, respectively.
In contrast, insufficient stirring power (0.015 W, Comparative Example 1 [比較例1]) results in
uncontrolled crystallization, producing platelike crystals that form irregular aggregates. This
material exhibits inferior electrochemical performance: 214.0 mAh/g discharge capacity and
capacity retentions of 88%, 83%, and 71% at 0.5 C, 1 C, and 3 C rates, respectively.
Excessive stirring power leads to particle fragmentation, as seen in Comparative Example 2 [比較例2]
(0.203 W) and Comparative Example 3 [比較例3] (0.685 W), resulting in non-spherical agglomerates.
The material's performance suffers due to poor morphology control and fragile grain boundaries.
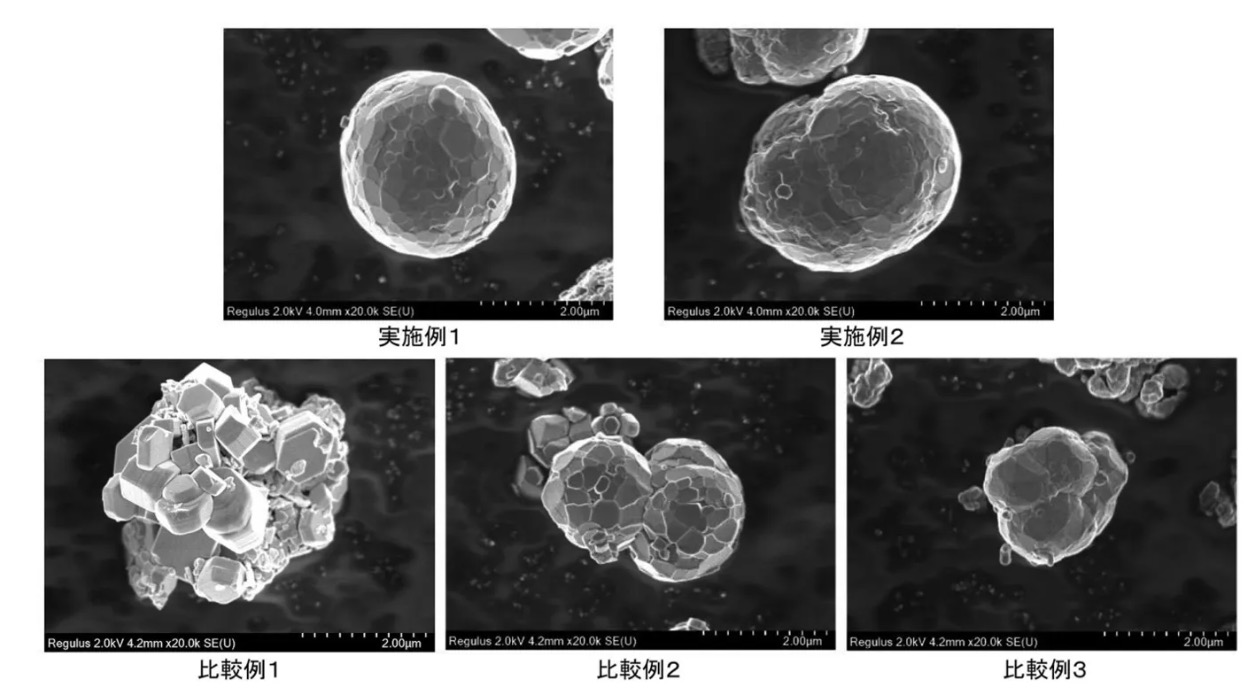
This work illustrates how careful optimization and control of the precursor solution stirring rate allows for particle morphology control
when fabricating LRLO (Li-rich layered oxide) active materials.
No cycling stability data was identified.
-
The premium version includes another two patent discussions, plus an Excel list with 50-100 commercially relevant recent patent families.
-
Fuel cells (PEMFC / SOFC / PAFC / AEMFC) – electrochemically active materials
-
A first ePTFE (microporous PTFE, polytetrafluoroethylene) membrane as first porous support (mass per area: 4.1 g/m2,
thickness: 12 μm, density: 0.4 g/cc, bubble point: 380 kPa) was hand-strained and fixed
in a metal frame. A first perfluorosulfonic acid (PFSA) solution in
water / ethanol was coated onto a PET-based polymer sheet
substrate. The ePTFE membrane was laminated to the wet coating, allowing PFSA
impregnation into the pores, followed by drying (160°C).
A second ePTFE membrane as second porous support (mass per area: 0.7 g/m2,
thickness: 6.6 μm, density: 0.1 g/cc, bubble point: 0.7 kPa) was similarly prepared. A
second PFSA solution in water / ethanol was coated onto the first dried composite, followed by lamination of the second
ePTFE membrane and drying (160°C).
For the cathode, a solution comprising PFSA, Pt/carbon catalyst (TEC10EA50E, Tanaka Kikinzoku Kogyo),
n-propanol / water was applied using a slot die. The coating wet thickness was 72 μm
at 0.5 m/min speed. After drying (60°C, 4 min), a cathode with ≈11 μm thickness and
0.41 mg-Pt/cm2 loading was obtained.
The polymer sheet substrate (releasable backer layer) was removed from the composite
electrolyte membrane. For the anode, a solution comprising PFSA, Pt/C catalyst,
n-propanol / water was
applied using a slot die. The coating
wet thickness was 15 μm at 0.8 m/min speed. After drying (60°C, 4 min), an anode with
0.11 mg-Pt/cm2 loading was obtained.
As shown in the Figure, SEM analysis reveals that while cracks (45) form in the
cathode layer (30), the reinforced electrode layer (37a) comprising the ePTFE membrane
infused with catalyst and ionomer remains intact.
27: reinforced electrolyte layer
28: first unreinforced electrolyte layer
29: second unreinforced electrolyte layer
30: cathode layer
37: reinforced electrode layer
37a: reinforced electrode layer in crack volume
45: crack
50: reinforced portion
100e: composite electrolyte membrane with electrode
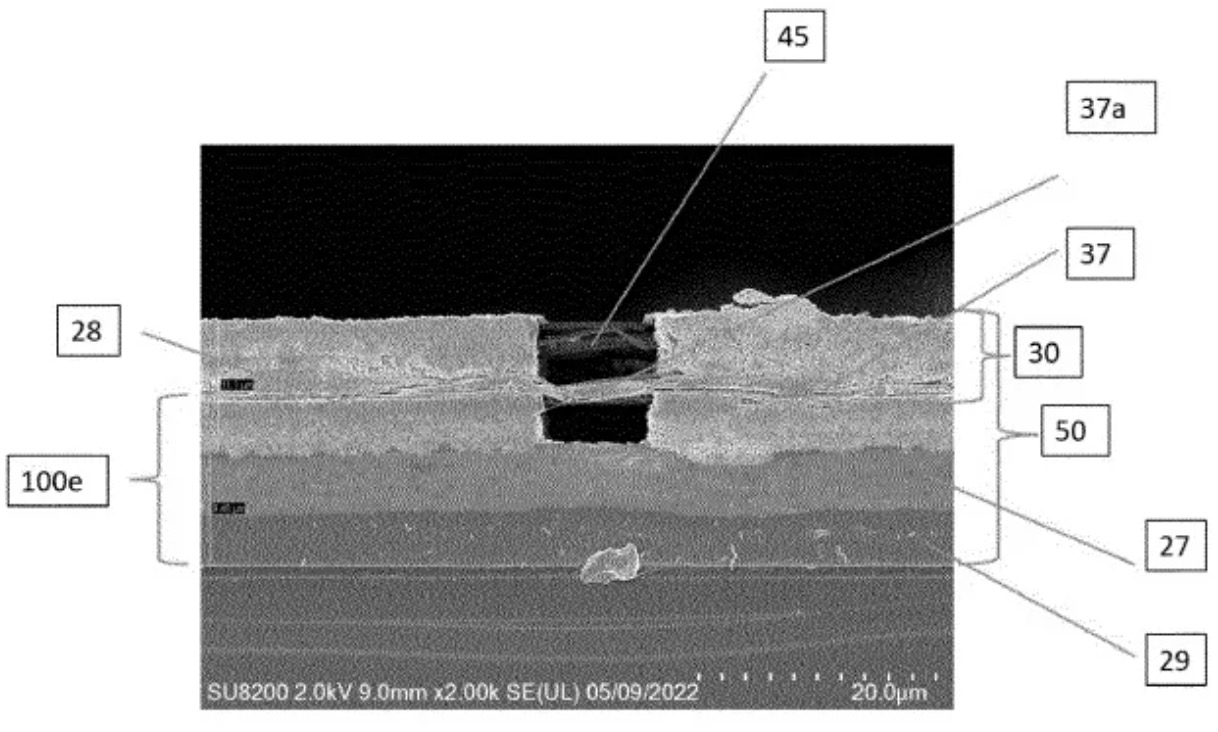
This work illustrates how membrane electrode assembly (MEA) resistance against crack formation can be improved by employing 2 layers based on expanded PTFE (ePTFE)
grades with different pore size distribution.
-
The premium version includes another two patent discussions, plus an Excel list with 50-100 commercially relevant recent patent families.
-
Triweekly patent lists for other categories (Excel files are included for premium users)
-
- Lithium metal containing batteries (excluding Li-S, Li-Air): XLSX
-
- Lithium-ion batteries – electrolytes – liquid: XLSX
-
- Lithium-ion batteries – separators: XLSX
-
- Lithium-sulfur batteries: XLSX
-
- Metal-air batteries: XLSX
-
- Na-ion batteries: XLSX
-
Prior patent updates
-
2024-12-03
-
2024-11-12
-
2024-10-22
-
2024-10-01
-
2024-09-10
|