-
This 'Deep Dive' was delivered to premium users (category: Li-ion battery cathodes):
-
'Recent Patents & Innovations in Lithium Manganese Iron Phosphate (LMFP) Cathode Materials'
Check out the preview.
-
Lithium-ion batteries – electrolytes – solid & semi-solid
-
A composition containing Li6.4La3Zr1.4Ta0.6O12 (LLZTO) powder, Li3N (sintering aid),
polyvinylbutyral-based binder BH-S (possibly by Sekisui Kagaku Kogyo), Hypermer KD-6 dispersant (Croda Industrial Specialties),
and dibutyl phthalate plasticizer was
prepared in NMP (N-methylpyrrolidone) / toluene solvent. This mixture was stirred (3,000 rpm, 5 min). The coated layer underwent
light exposure treatment using a PulseForge system (300 V, 3 ms pulse duration, 10 pulses, 62% duty cycle, 20 Hz, 100 repetitions)
to achieve 600°C sintering temperature, resulting in a fused, porous layer (≈23 μm thickness, see SEM image below).
This porous structure was infused with a polymer electrolyte composition based on trimethylolpropane ethoxylate triacrylate (TMPETA, 5 mass%),
t-butyl peroxypivalate (t-BPP, 1 mass%),
LiTFSI (16.9 mass%), LiDFOB (2.1 mass%) and LiPF6 (0.6 mass%) in EMC (50.7 mass%) and FEC (23.7 mass%).
For electrodes, artificial graphite negative electrodes were prepared using graphite, carbon nanotubes (CNT), and SBR binder
(92:5:3 mass ratio) coated on 15 μm Cu foil. Positive electrodes contained Li[Ni0.6Co0.2Mn0.2]O2,
carbon black and PVdF (92:5:3 mass ratio) coated on 15 μm aluminum foil and dried at 130°C.
SEM imaging (도5a, 도5b) showed a porous structure with 18% porosity and 23.32 μm thickness for the composite electrolyte layer.
EDS analysis confirmed removal of carbon content after light exposure sintering, while sulfur was detected in oxide electrolyte pores,
indicating successful polymer electrolyte penetration.
Full cells with graphite-based negative electrodes and NMC622-based positive electrodes exhibit a first discharge capacity
of 180.6 mAh/g, a first cycle coulombic efficiency of 90.8% and 92.6% capacity retention after 100 cycles (0.5 C charge / discharge).
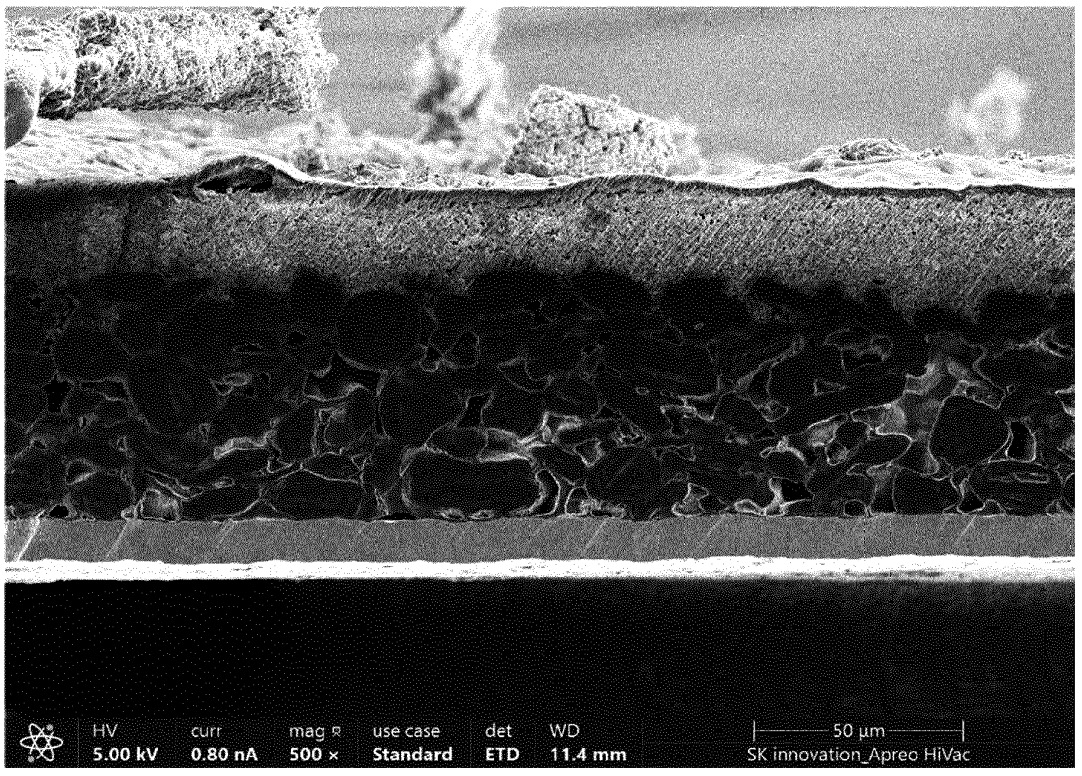
This work illustrates how porous sintered garnet oxide layers can be obtained through light sintering at 600°C.
Presumably, the reduced sintering temperature as compared to processes carried out at >1,000°C might allow for cost
savings (reduced energy consumption, faster throughput rate), at the expense of obtaining 'less perfect' oxide layers.
By infusing the oxide layer 'with the right polymer' (that exhibits favorable ionic conductivity and prevents Li-ion dendrite formation),
a well-balanced electrolyte layer might be achieved in terms of balancing performance, costs and safety. Because the polymer
layer is hosted inside the porous structure, it does not need to form a free-standing film by itself (relaxed processing requirements).
It will be interesting to see performance data with lithium metal electrodes and with polymers or gels that do not contain liquid
carbonate electrolytes.
-
The premium version includes another two patent discussions, plus an Excel list with 50-100 commercially relevant recent patent families.
-
Lithium-ion batteries – negative electrode (excluding Li metal electrodes)
-
A method for preparing silicon-carbon composite negative electrode materials for lithium-ion batteries was developed based on mixing two
Si-carbon composite active materials with different D50 particle sizes,
with comprehensive characterization shown in particle size distribution plots, cross-sectional SEM imaging,
and performance data tables (see Figures).
Among all samples tested, sample C3 demonstrates superior performance, achieving 965 cycles while maintaining 80% capacity retention
(full cells with NMC-based positive electrodes, 1 C charge / discharge).
The particle size distribution for this optimal formulation is shown in the top Figure (mass fraction: 0.75, alpha value: 4.99).
Mass fraction: mass fraction of Si-carbon composite precursor with larger particle size
Alpha value: D50 size ratio between Si-carbon precursors with large and small particle sizes
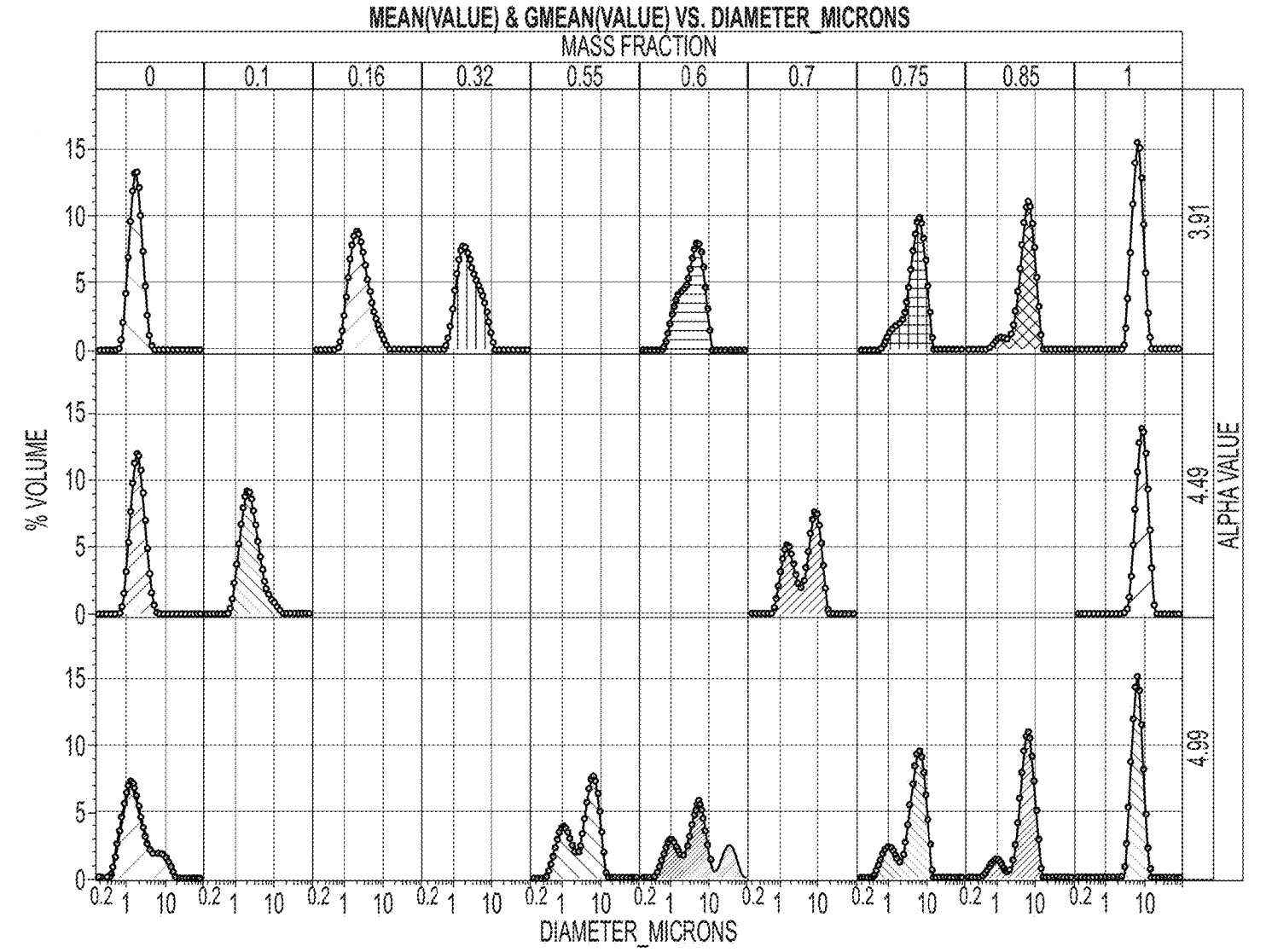
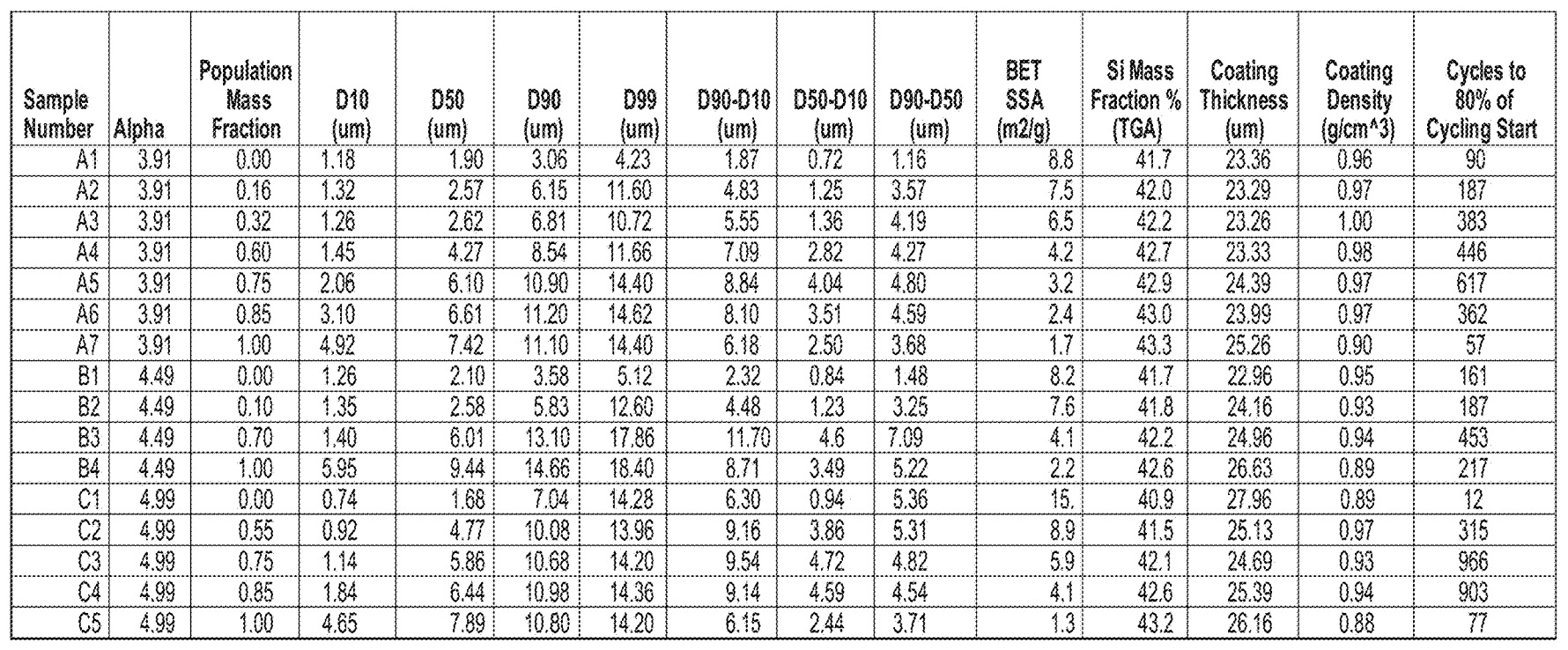
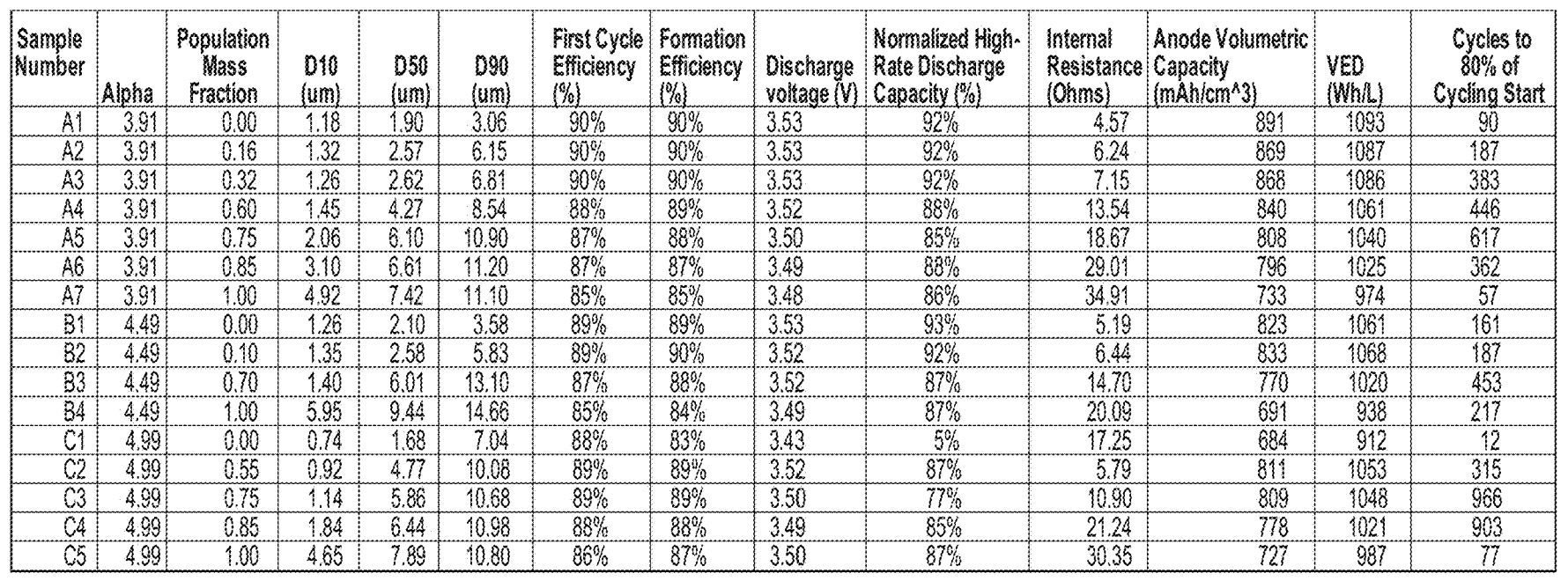
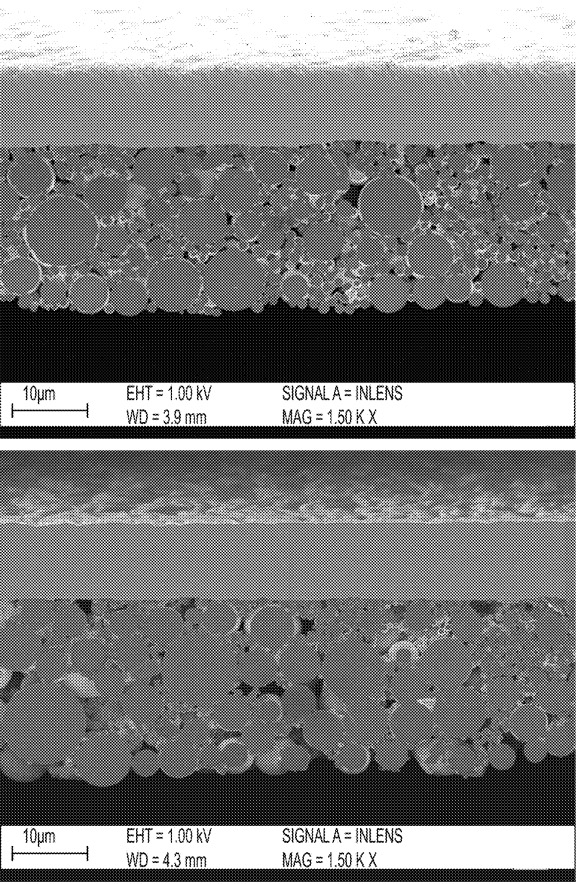
This work illustrates that a pronounced bimodal particle size distribution allows for favorable cycling stability at a favorable volumetric energy density.
The BET specific surface area of 5.9 m2/g is higher than for typical graphite-based electrodes, but appears to still be low enough
as not to cause aging due to excessive SEI formation in combination with liquid carbonate-based electrolytes.
-
The premium version includes another two patent discussions, plus an Excel list with 50-100 commercially relevant recent patent families.
-
Lithium-ion batteries – positive electrode
-
A composite transition metal hydroxide precursor Ni0.95Co0.03Mn0.02(OH)2 (D50: 3.5 μm) and LiOH were mixed
(molar ratio transition metals : Li = 1 : 1.05) and heat-treated (850°C, 6 h, oxygen atmosphere). After pulverization, secondary firing was conducted
(750°C, 9 h, oxygen atmosphere) to form single-particle lithium composite transition metal oxide.
The oxide was mixed with Co(OH)2 (molar ratio 98 : 2) and Al(OH)3 (500 ppm) using an acoustic mixer, followed by a heat treatment (700°C, 5 h).
The resulting cake was pulverized to obtain a powder.
The powder was mixed with B(OH)3 (molar ratio 99.75 : 0.25) and heat-treated (330°C, 5 h).
In half-cell tests, the material exhibits a discharge capacity of 215.7 mAh/g with 87.2% first cycle efficiency and a DCIR of 23.2 Ω,
compared to 211.8 mAh/g, 86.7% and 22.4 Ω for a B-free comparative material. Under accelerated testing conditions
(45°C, 0.5 C charge / 1.0 C discharge), the material maintains a capacity of 95.3% after 50 cycles with a resistance increase of 157.2%,
compared to 95.1% and 162.3% for the B-free comparative material.
This work suggests extensive optimization work was done to identify a B / Co / Al-rich coating
applied in two steps for a high-nickel NMC material. The two-step coating process allows for fine-tuning elemental content
right at and just below particle surfaces.
-
The premium version includes another two patent discussions, plus an Excel list with 50-100 commercially relevant recent patent families.
-
Fuel cells (PEMFC / SOFC / PAFC / AEMFC) – electrochemically active materials
-
A carbon-supported platinum-nickel alloy catalyst was prepared in multiple steps:
A carbon nanotube support was dispersed in ethylene glycol using a homogenizer (4,500 rpm,
30 min). The dispersion was passed through a porous cell (200 μm) under high pressure to
control particle size distribution.
The dispersion and platinum precursor (such as PtCl4) were mixed in a reactor (200 rpm, 30 min), heated
(160°C, 2 h) to deposit platinum nanoparticles within the carbon support pores.
The cake-like platinum catalyst was mixed with a nickel precursor (such as NiCl2) in water / ethanol solution
(1 h). The mixture was concentrated in a rotary evaporator (40°C, 200 mbar, 70 rpm) to
evaporate ethanol, followed by water evaporation (75°C).
Remaining organic impurities were removed under vacuum (150°C, 200 mbar), followed by chemical
alloying of platinum and nickel (800°C, 200 mbar). The catalyst was stabilized in
water / nitric acid / acetic acid solution (30 min), then stirred (90°C, 6 h).
The product was dried under vacuum (120°C, 200 mbar,
24 h), and ground (30 s) to obtain a uniform powder. The resulting platinum-nickel catalyst A
exhibits an average crystallite size of 3.20 nm and a superior initial MEA (membrane electrode assembly) activity of ≈1.35 mA/cm2 at 0.6 V,
as compared to
≈800 mA/cm2 for the commercial platinum catalyst TKK from Tanaka Corp. (see Figure).
A TEM (transmission electron microscopy) analysis confirms uniform dispersion of alloyed
nanoparticles on the carbon support without agglomeration (see Figure).
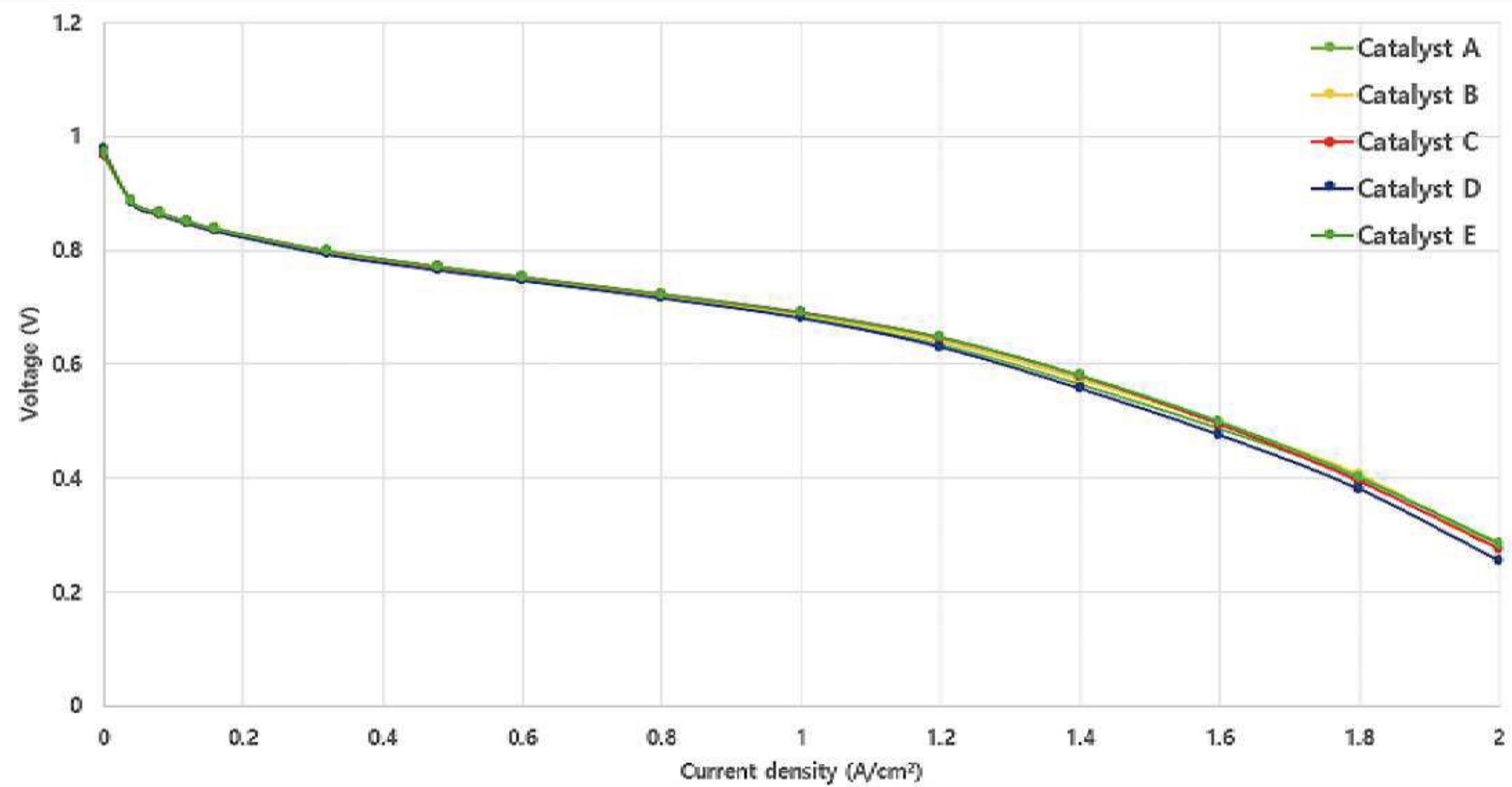
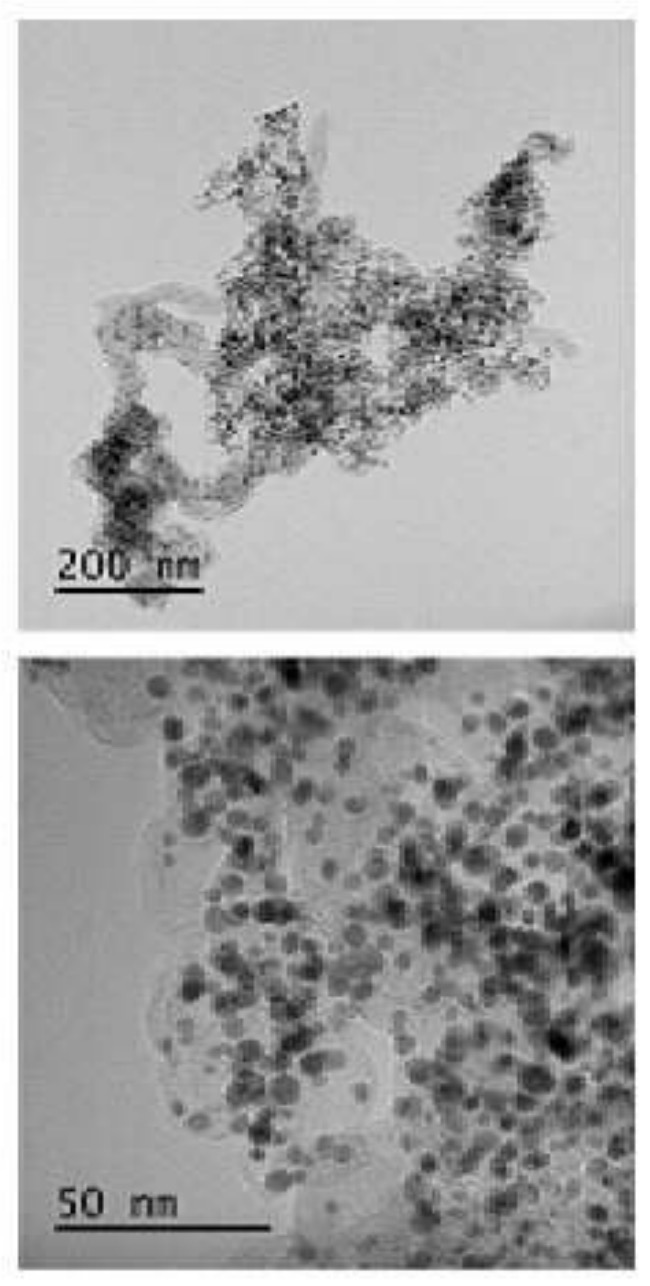
This work illustrates favorable performance for a Pt-Ni catalyst in which Pt and Ni precursors were first mechanically mixed and then chemically alloyed
and converted to a metallic alloy through a high-temperature treatment.
-
The premium version includes another two patent discussions, plus an Excel list with 50-100 commercially relevant recent patent families.
-
Triweekly patent lists for other categories (Excel files are included for premium users)
-
- Lithium metal containing batteries (excluding Li-S, Li-Air): XLSX
-
- Lithium-ion batteries – electrolytes – liquid: XLSX
-
- Lithium-ion batteries – separators: XLSX
-
- Lithium-sulfur batteries: XLSX
-
- Metal-air batteries: XLSX
-
- Na-ion batteries: XLSX
-
Prior patent updates
-
2025-12-23
-
2024-12-03
-
2024-11-12
-
2024-10-22
-
2024-10-01
|